the web of science
Nancy Marie Brown
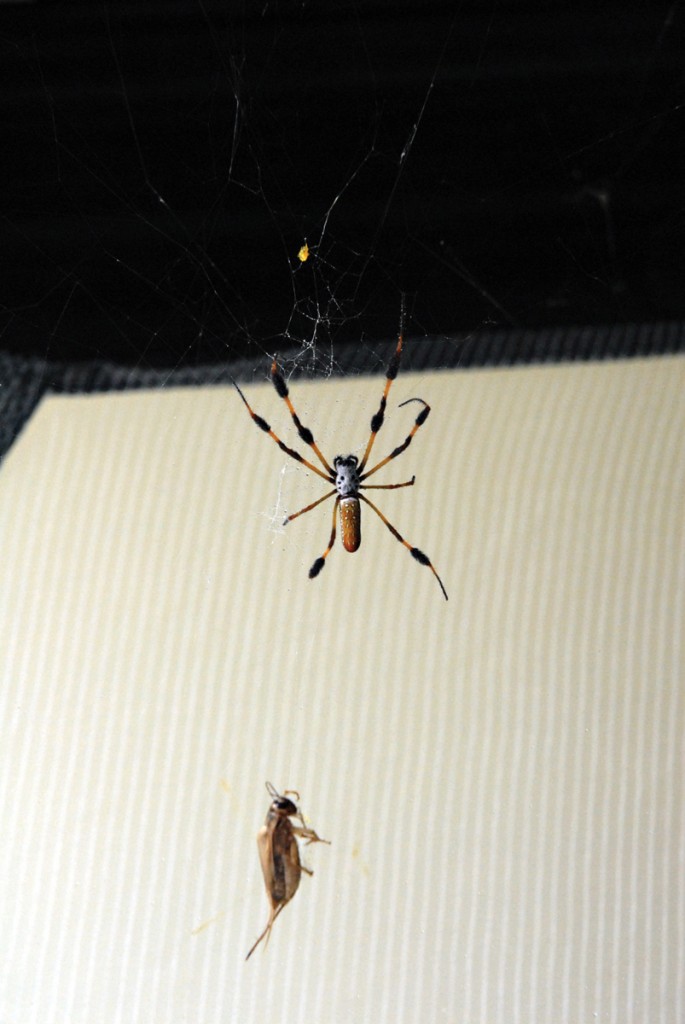
In Michael Ellison’s spider tent, a golden orb weaver (Nephila clavipes) stalks prey trapped in a silken web. For researchers, the silk itself is the quarry. Photo by Craig Mahaffey.
In the depths of Sirrine Hall is a screen tent full of spiders. At least, it should be full of spiders. When materials scientist Mike Ellison peeks inside, on a day in November, he finds only one. “Looks like we’ll have to catch some more,” he says, demonstrating his swooping technique, jar in one hand, lid in the other.
He can’t just pick them up; golden orb-weavers are too delicate. “I love to go out and collect them,” he says. “It’s fun.”
An expert in polymer fibers, Ellison and his graduate students have perfected a technique of “milking” the spiders to collect their silk. He pops the lid off a pharmacy pill bottle to expose a homemade silk-collecting reel—it looks like a tiny fishing reel—and tilts it back and forth. A few strands of spider silk glisten like gold as they catch the light. Otherwise they are invisible.
And 800 times stronger than steel.
As a fiber, Ellison points out, spider silk is pretty remarkable. Strong when heated or frozen, and when wet, it contracts dramatically. It conducts both heat and light. It’s also biodegradable, naturally. Since the 1970s, scientists have imagined making everything from bulletproof vests to parachute cord to computer chips to medical sutures and vascular grafts out of spider silk.
But no one so far has identified the structural traits that give this extraordinary fiber its properties.
Ellison believes part of the secret lies on the surface of the silk. He dreams of making a synthetic mimic. So he milks his golden orb-weavers and sends his collecting reels over to Rhodes Annex, to Delphine Dean’s Multiscale Bioelectromechanics Lab.
Dean is an expert in visualization at the nanoscale—in other words, she can see things so small as to be almost invisible, such as the individual amino acids on the skin of spider silk.
“Spider silk is really interesting stuff,” says Dean, a bioengineer. A single golden orb-weaver can make seven kinds of silk, she notes, while spiders of other species make silk with different properties. “Which circumstances make those different characteristics?” she wonders.
To find out, she, colleagues Ellison and Molly Kennedy, and a team of Creative Inquiry students—two undergraduates from materials science, one from bioengineering, and materials science graduate student Benoit Faugas—take a tiny sample of dragline silk milked from a golden orb-weaver and examine it with an atomic force microscope. “We are looking at the surface, not at the whole composition of the fiber,” Dean explains. Spider silk, like wool or human hair, is a protein, a chain of amino acids. “We’re not so much interested in what the fiber is made of,” Dean says, “as much as in learning which amino acids are on the surface.”
It’s a technique Dean devised for an earlier collaboration with Ellison and Kennedy on wool. She also uses atomic force microscopy to study teeth, cardiovascular muscle cells, the effects of radiation on cartilage, how cells respond to growth factors, and the surface properties of dirt. Why so many projects? “I don’t like being bored,” she laughs.
She mentors still more projects: medical training simulators, a mind-controlled robot, the use of ultrasound for rotator cuff injuries, exhibits for the Roper Mountain Science Museum, and the design of medical devices for developing countries. One of these last projects brilliantly transforms an old inkjet printer into a device for making diabetes testing strips.
All told, Dean is responsible for one postdoctoral fellow, four Ph.D.’s, three master’s, and forty-six undergraduates, in eight different teams. “Creative Inquiry is cool,” she says of the Clemson-funded research program. “You get a team of undergraduate students, give them an idea, and let them run with it. Once you have a team, the senior members help train the new students, so it’s also a great opportunity for graduate students to learn how to mentor.”
Explaining these projects in the lab, Dean uses expressive arm gestures—the byproduct of years of figure skating and studying ballet. In the elevator, on the way down to demonstrate the atomic force microscope in the Rhodes Annex basement, she unselfconsciously takes up the ballerina’s first position, her feet making a perfectly straight line, heels together, toes 180 degrees apart. Last Christmas, she danced, en pointe, in The Nutcracker, something she likes to tell school children when she’s talking to them about science. “It’s important for little girls to know that you can be a bioengineer and still wear a pink tutu,” she says. “You can be a scientist and an engineer and still have a life outside of work.” Her husband, computer science professor Brian Dean, she points out, is a pianist, a runner, and a cook.
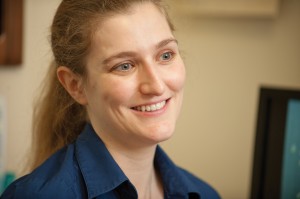
Delphine Dean: “I like research, but you can do research in a lot of different venues, in government, in industry. What I really like is mentoring students. I like to see what ideas they come up with.” Photo by Craig Mahaffey.
Born in France, Dean came to the U.S. with her family when she was eight and was immediately thrown into the public school system, though she knew no English. Even then she was a fast learner. “I arrived in February. By June I was fluent in English.” She traces her interest in bioengineering to two events. “My sister had a meniscus tear when she was in sixth grade and got arthroscopic surgery. I found that fascinating. I knew I didn’t want to go into medicine, but I wanted to study cartilage.” The other impetus was a toy robot. “I thought, ‘Wouldn’t it be cool if people who were missing limbs could have a prosthetic that basically was a robot controlled by their body?’
“But I like working with people too, so I love it when people come to me with ideas. The American public needs to know that scientists don’t just sit at a bench in isolation. I think I prove that’s not how it works. You can’t solve problems all by yourself. You need a team. Why I went into academia was to mentor. I like research, but you can do research in a lot of different venues, in government, in industry. What I really like is mentoring students. I like to see what ideas they come up with. It’s fun. But the best part of training the new crop of scientists and engineers is that you can actually see them becoming independent researchers. They will come up with something that surprises you, something that you’d never think of yourself.”
Her collaboration with materials scientist Molly Kennedy and her students is a case in point. The third member of the spider-silk team, Kennedy usually studies nanocomposites—materials made of two or more substances combined at very small scales, such as wear-resistant or radiation-resistant coatings. Her expertise is in determining how the fine-scale structure of a material controls its properties.
She met Dean in 2008. “I had a gifted grad student, Bonnie Zimmerman, who wanted to work on biocomposites,” Kennedy explains, “so we walked over to Delphine Dean’s office. She said, ‘What do you think about teeth? A lot of people have studied bone, but few have studied teeth.’”
They put together a Creative Inquiry team to examine what happened to the structure of a tooth when it was whitened, for example, by using a whitening toothpaste. They found, to their surprise, that whitening not only changes the surface of the tooth but also affects the structure of the dentin deep inside. Depending on the whitening process, it makes the enamel stiffer, and so slightly more likely to crack, while at the same time making the dentin inside the tooth stiffer in one case and more elastic in the others.
“Research is exciting,” Kennedy says, “but it’s not about going into the lab unprepared. Ninety percent of it is knowing what other people have done before you and using that information to create what we call a design map, a prediction of what will happen. It doesn’t always work, though. We didn’t think whitening would affect the dentin. That was a surprise.”
They repeated the experiment and had the same results. Then students and professors talked it over and tried to develop a new hypothesis to test. They published their results in 2010.
“My job as an academic is not to produce papers,” Kennedy says, “but to bring in and educate students to be better than me. That’s what my dream job is. And here at Clemson, we can really focus on the students.” In her collaborations with Dean, she says, “We’re trying to create students who are well-rounded—students who have substantial knowledge in their home fields, but who can also work in related areas.”
But what benefits the students also helps the professors, Kennedy says. “Students really aid our research, because they think in uninhibited ways. They aren’t restricted by their expectations, by what we call investigator bias. It’s also nice in a lot of ways to have a colleague from a different department. There’s a synergy. Delphine can understand what the cells are doing, and I can understand the structure.
“But what I like about Delphine most,” Kennedy adds, “is that she likes to educate and strengthen her collaborators. You not only get a chance to collaborate, you get a chance to grow as a researcher.”
Dean, Kennedy, and Ellison and their students began working together several years ago. In 2011 they published the result of a very practical study of wool fibers, funded by Kentwool, a local company, with the goal of making wool clothing washable.
Wool shrinks because of its structure. Under a scanning electron microscope (SEM), a wool fiber looks scaly, like fish skin. On the wooly coat of a sheep, the scales don’t tangle since they all face the same direction. Instead they serve the useful purpose of removing dirt from the animal’s skin. Yet when the wool is spun into thread and woven or knitted into cloth, the scales are turned every which way. They catch on each other. In a washing machine, they ratchet closer together and the cloth shrinks. Taken to extremes, this process produces felt.
But wool treated to make it washable sometimes has a slimy feel. Worse, the standard chemical treatment isn’t environmentally friendly. It produces high levels of pollutants—organic halides—in the wastewater.
Since wool is a protein, made up of chains of amino acids, theoretically enzymes could be used instead of chemicals to eat away the rough edges of the scales and inhibit the felting process. But unless they target just the scales, the enzymes also weaken the wool fibers. Few enzyme processes have yet met the industry’s standards of less than 10 percent shrinkage in a washing machine along with less than 10 percent loss in fiber strength.
“But there are enzymes, or proteases, that are specific for particular amino acid sequences,” Ellison notes. “They will remove amino acids only if they are in a particular arrangement. So our idea was, if we could map the sequence of amino acids on the surface, we could design a protease to remove only the amino acids that are on the ridges of the scales, and leave the rest of the wool alone.
“The idea was phenomenal,” he says, “but our results weren’t. There was not a real clear line of amino acids along the ridge. We had some luck with a protease, but it wasn’t great. And we couldn’t scale it up fast enough for our research sponsor. Given the intense competition in this business, they quit funding us—it was a business decision—and we went on to other things.”
Namely, spider silk.
Ellison became interested in spider silk some ten years ago, looking for a way to make better vascular implants. “I was at a conference listening to a talk about polyester tubes used as vascular implants. I thought what we needed to do was to be able to make a tube using the person’s own DNA, so I wanted to look at recombinant-DNA methods of making proteins that would form fibers. I was playing music together with a friend of mine who is a molecular biologist—we have a band and play rock-and-roll, country, swing, and just general old Americana together—and he suggested I model the fibers after spider silk. I graduated several students out of that program, but I didn’t achieve the Holy Grail of making spider silk.”
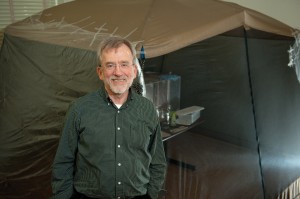
Michael Ellison at the spider tent. Ellison switched from wool to spider silk after a musician friend suggested it. Photo by Craig Mahaffey.
Working with Dean and Kennedy he hopes to learn more about the structure of spider silk, with the idea of mimicking it, not making it. The three researchers have submitted a proposal to the National Science Foundation.
“When you’re a materials scientist,” Ellison explains, “you study how process influences structure and gives you the resulting properties. That’s the triangle: process, structure, properties. Now, people have analyzed spider silk for its amino acid content, and they have discovered most of the sequence, but when you do amino acid sequencing, it’s a bulk study. You dissolve the protein.” The results don’t reveal which amino acids are on the surface of the fiber and which are inside.
Ellison, Dean, and Kennedy believe knowing this is the key. “Nobody has distinguished the chemistry of the skin of the silk from the rest of it,” Ellison says, “because the fiber is so small. But one of the tenets of materials science is that the surface contributes mightily to the properties of the material. It’s the idea of there being an interface between the material and the outside world.” Using the atomic force microscope, they hope to better understand the surface of spider silk, especially how its structure relates to the silk’s properties. “Then we will better understand what leeway we have in trying to create a similar structure,” Ellison says. “We’ll know how close we have to fake it to still get a good fiber.
“Making spider silk is a ridiculously complicated process,” he adds, “and the spider has about a million years’ head start on us.” In the spider’s abdomen is a gland connected to a long tube. A protein is created in the gland, and by the time it has traversed the tube it is a silk fiber that the spider can extrude through its spinnerets.
“What goes on in between the gland and the spinneret is very, very complicated,” Ellison says. “The spider is changing the pH, removing the water—and it’s a self-assembly system. It doesn’t take a lot of energy, though she does have to eat. If we could mimic that,” says Ellison, “if we could learn to make a strong, light fiber with the remarkable properties of spider silk under ambient conditions, well, it would be really cool.”
How to see the invisible
Delphine Dean’s skill with atomic force microscopy is key to the spider silk study. “You may have seen pictures from a scanning electron microscope,” Dean says. “An SEM can image a whole bug, and then zoom in on its eye. With the AFM, we’re more limited.” The largest thing an AFM can look at is 100 microns in the X-Y, or back-and-forth, direction and 40 microns in the Z, or up-and-down, direction. “A strand of my hair is seventy microns thick,” Dean says. Her adviser in graduate school, she says, “had gorgeous thick hair. It was one hundred microns in diameter. So that gives you an idea of the scale.” A spider is too big for the AFM. But spider silk, at only 5 microns in diameter, is a perfect subject.
A relatively new scientific imaging technique, the AFM was invented in 1986 and commercialized in 1990. Dean was a junior at the Massachusetts Institute of Technology when MIT bought one of its first ones in 1999. Because AFM was so new, the faculty brought together an interdisciplinary team to, as Dean puts it, “see what it could do.” The team included Dean, then a student in electrical engineering. “I was never bored,” she says. She went on to do a Ph.D., studying cartilage with the AFM, looking at how the molecules that make up cartilage interact and especially how those interactions change with age to cause arthritis. “I had to learn how to set up the AFM and how to use it.”
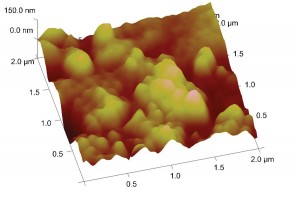
Atomic force microscope image of the surface of spider silk. Photo courtesy of the Delphine Dean lab.
Explaining it to her students now, Dean compares the microscope to a record player: “A needle is dragged along a surface and moves up and down along the bumps and grooves.” She shows them a picture of a turntable. “When I’m done, they tell me a record player works like an AFM!” Who has a record player these days? In this analogy, the record or LP is the sample to be tested. The needle is the probe tip that scans across the surface of the sample. The arm of the record player is the tweezer-like cantilever that holds the probe tip. But instead of turning the wiggles of the arm into music, the AFM turns the wiggles of the cantilever into an image by shining a laser onto it and measuring precisely how the cantilever dips and bobs. The result is a 3-D image of the surface topography.
“It’s not an improvement over SEM, just a different imaging modality,” Dean explains. To look at something under a scanning electron microscope, you must first coat your sample with carbon, gold, or some other metal, then place it inside a vacuum. “Because the AFM is all mechanical,” Dean says, “you can do it in water or under physiological conditions, using living cells and tissues. You can characterize just the surface, or you can watch the tip interact with the surface. For example, we use the AFM as a very tiny mechanical tester to see how stiff or soft a cell is. Or, if you put a protein on the probe tip, you can look for the matching protein in your sample. You keep dipping the tip down until you feel it stick. You come down, you catch something, and you can pull on it. You can make a protein unwind and get an idea of the length of the protein or how elastic it is. If you know what’s on your tip and you watch it interact with your sample, you can map the functional groups on the sample surface—though if the surface is really sticky, it’s hard to do anything because the probe tip gets stuck.”
Dean’s lab at Clemson, the Multiscale Bioelectromechanics Lab, now has two AFM machines. The one upstairs in Rhodes Annex sits on a thick granite slab and is encased in a black protective housing the size of a large refrigerator. But most of the equipment inside the casing belongs to an optical microscope that lets you watch what the AFM is doing. “It looks impressive,” Dean says. “But all the impressive parts are not the AFM. I remember back at MIT when the public information office sent people to the lab to take a picture of their new machine. They were so disappointed. It was so small. Nothing like an SEM, which is huge.”
Small and also temperamental. “Sometimes you turn it on and it’s not in a good mood and you get nothing,” Dean says. “We have a love-hate relationship. It gets you cool data, and a lot of data, but it’s a finicky machine.”
Dean opens a small plastic case and points to a sliver of metal about the size of a fingernail paring. “This is the cantilever on top of which is the probe tip. It’s a tiny, tiny probe, too small to see with the naked eye. How the heck do you put a protein on this probe tip so it will stick? I had to learn some chemistry really fast. So I talked to someone in chemistry who told me, ‘We use this to stick molecules to gold.’ I tried it and it worked.” But it takes good eyesight to then properly mount the tiny probe tip and the delicate cantilever that guides it, like the arm of the record player, across the surface. “I can’t have too much coffee before I do this,” Dean says, “or I can’t get the tip in there.”
Then there is the problem of vibrations. Even with the AFM sitting on a sturdy granite slab supplied with shock absorbers—or, for the AFM in the Rhodes Annex basement, on a cushion of air continuously pumped from a canister—vibrations can ruin the data. “When we’re doing surface characterizations, and we’re looking at nanoscale differences,” Dean says, “you can’t even talk in the room while you’re taking measurements. If I clap my hands, you’ll see it in the data.” If you turn on the ceiling fan, you’ll get wavy lines. Forget about sneezing. The building construction on campus is also a problem. “There’s only so much you can do while they’re jackhammering. The tip goes all over the place.”
Delphine Dean is an assistant professor of bioengineering, Michael S. Ellison is a professor of materials science and engineering, and Marian (Molly) Kennedy is an assistant professor of materials science and engineering, in the College of Engineering and Science.