Carbon’s magic carpet
Neil Caudle
In the material world, an ethereal skin of pure carbon can deliver a jolt.
If you set fire to this page, a flake of ash might rise and float away. That little smidgen of carbon, as black as the wing of a crow, is so fragile it crumples to dust when it lands in the palm of your hand. But carbon is also the stuff of a diamond, which is eternal (or so we say, when we propose), and hard enough to sever steel.
Between these extremes, meet Apparao (Raja) Rao.
Rao is a physicist, which most of us are not. But he is, like all of us, a walking contradiction, a carbon-based life form akin to both diamond and ash. Rao is adept with contradiction. In his lab, he can make the flimsy strong, the rigid pliant, the bulky small, and the ephemeral built to last. Almost every time Rao mows his lawn, drives his car, adjusts his thermostat, boots his computer, or reads the news, he imagines a new incarnation for carbon.
In the lab, Mehmet Karakaya, a graduate student from Turkey, lifts a blue-gloved hand to show me a swatch of black carbon so thin and ethereal that he might have just plucked it from the air. He scrunches it, wraps it over his thumb. The stuff is pure carbon, but it flexes and dimples and drapes like a skin.
I tug it with tweezers; it puckers but does not break. This is not diamond; this is not ash. This is something surreal from a realm in between.
But I am on the job today and therefore bound to ask, so what? What good is this thin little carpet of carbon? Can it carry us away?
In a manner of speaking, it can. Nanomaterials, Rao believes, have a nearly magical potential to support new industries, maybe even levitate an economy or two.
This is more than wishful thinking. Last year, the National Science Foundation (NSF) awarded Rao and his collaborators $1.2 million to find ways to scale up production on some of their nanomaterials, to make them practical for manufacturing. The goal: energy-storage devices that could pump up the power of batteries and capacitors in hybrid and electric vehicles, power tools, and various other products. And today’s industries, especially those working with renewable energy, could use a good jolt.
High energy, low power
The problem with current technology, Rao explains, is that while our batteries store lots of energy, the power we extract from them is relatively low, because the batteries cannot deliver their energy fast enough. Capacitors, which regulate flow, can release energy quickly but cannot store very much of it at once. So there’s a mismatch, a gap. And the gap has been holding back progress, especially in the use of renewable energy from sun and wind.
“When you are making power tools and hybrid vehicles, you need high power and high energy at the same time,” Rao says. “So that’s the goal of this project, to fill that gap.”
The gap-filling technology Rao and his colleague Mark Roberts, a chemical engineer, have in mind is a new kind of nanomaterial, one they have already made in the lab in small batches: a very thin layer of carbon, much like the film I tweezed, coated with a special kind of polymer, to hold and deliver a charge. With the NSF grant, they intend to crank it out in rolls.
“There has been a lot of talk on the amazing properties of nanostructured materials,” Rao says, “but if you go ask somebody for the nanomaterial, they’ll ask you how many milligrams you want. With milligrams you can’t do anything, right? So the NSF and other funding agencies realize this, and that’s why they’re funding grants that focus on scalability.”
But will the system Rao has in mind really work? He smiles, sits back in his chair. “Yes,” he says. “I think it will.”
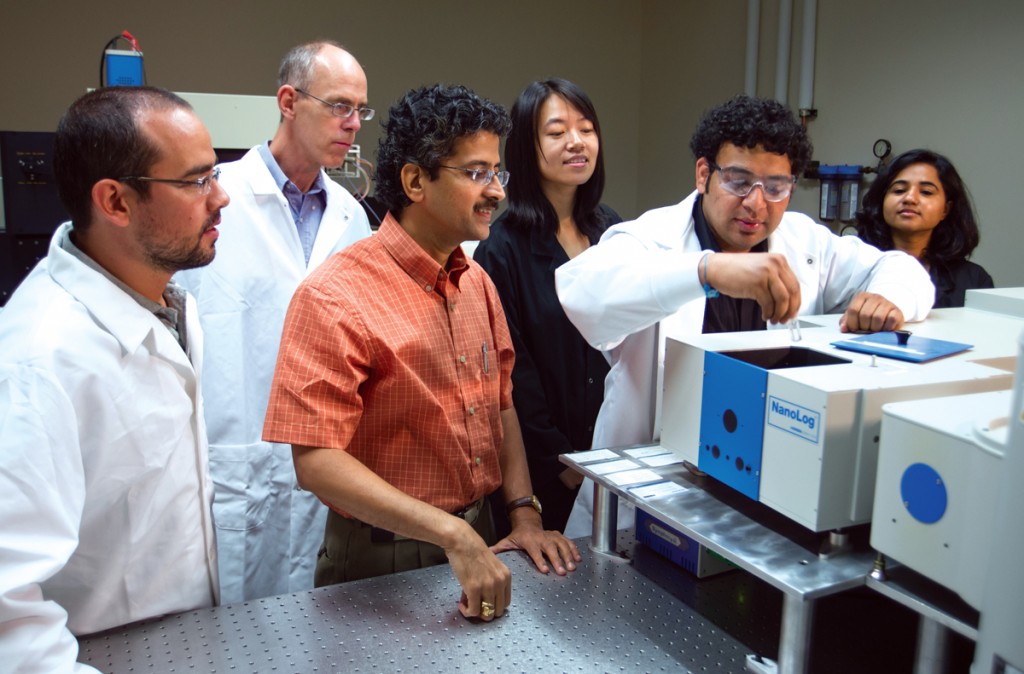
Apparao Rao (in orange) and members of his lab watch as Ramakrishna Podila (Rama) loads a cuvette with graphene quantum dots into a photoluminescence spectrometer. Others include (from left to right): Mehmet Karakaya, Herbert Behlow, Jingyi Zhu, and Deepika Saini. Rama and Rao’s work on the origin of photoluminescence in graphene quantum dots is featured on the cover of the October issue of Advanced Functional Materials. The quantum dots “glow” under ultraviolet light and have great potential for biomedical imaging. Photo by Craig Mahaffey.
A spin around the lab
Rao has several reasons for optimism, but we’ll come back to that later. For now, let’s take a spin around his lab. It is not very large, as Big Physics goes these days—three faculty members and six students—but the range of projects under way is daunting. The lab and its collaborators are pursuing dozens of potential applications in medicine, electronics, optics, sensing, energy, environmental protection, and more.
The list, to consider it now, seems outrageously ambitious. But Rao, a slender, soft-spoken man, doesn’t show the ambition; he passes most credit to colleagues and students. What I heard, when he described their work, was not brag but delight.
I want to know the source of that delight, so Rao begins to explain it. This requires some patience on his part, because physics does not readily peddle its marvels to tourists like me. For a physicist, the delight is in the details, in the unfolding story of the science. But for print, I need a punch line, a takeaway, a gist. So here, for the record, it is: Rao’s lab and its collaborators are creating new technologies that could advance high-tech industries, generate jobs, and help provide cleaner, cheaper energy. That’s the bottom line.
But I still want to know what makes the magic carpet fly.
The soccer ball that could
Rao begins the story with a toy-like model, a skeletal sphere. He sets it on his desk between us to help me consider its faceted structure, which, as he points out, is geometrically the same as a soccer ball: twelve pentagons and twenty hexagons. The toy is plastic, but the structure it represents is made of sixty carbon atoms, hence its scientific name, C60. The structure is also known as a spherical fullerene, or, more commonly, a buckyball, after the famous geometry of Buckminster Fuller’s geodesic dome.
An actual buckyball is small, about one nanometer in diameter, which is one billionth of a meter. This is a very strange neighborhood indeed, governed by the quirky laws of quantum mechanics, where a particle is a wave is a particle, where gold reveals its true color (pink), and where buckyballs breathe like pufferfish.
Scientists like Rao make fullerenes in the lab, but they also occur naturally, even in outer space. And they are incredibly tough. “The bonds in the carbon are what we call covalent bonds,” Rao says. “They are very, very strong.”
For Rao, the buckyball was generation one. He worked on it through his early career, at the University of Kentucky, and wrote a book on the subject with his mentor, the late Peter Eklund. Today, buckyballs are famous, proving useful as ball bearings for tiny machines, for example, or as cages for safely transporting heavy elements that fluoresce in the body, to pinpoint disease.
But the buckyball was just the seed. The tree was still to come.
Generation two: the nanotube
Rao took the mesh-like structure of the fullerene and wrapped it into carbon nanotubes. He was not the only person doing this kind of thing. By the year 2000, when Rao arrived at Clemson, carbon nanotubes were among the hottest new topics in science, and labs around the world began cranking them out. Nanotubes, the stiffest and strongest materials ever discovered, soon found their way into carbon-fiber baseball bats, golf clubs, and auto parts. But salting the world with nanotubes posed some risks, especially when the tubes escaped in the flows of industrial waste. In the environment, nanotubes quickly acquired scummy coatings of organic matter and began to masquerade as food for microbes. Making their way up the food chain, nanotubes concentrated in the tissues of fish and other animals, where they proved to be toxic to cells.
Rao, working with Pu-Chun Ke in biophysics and Hong Luo in genetics and biochemistry, has shown how this works, in both animals and plants. Rice plants, Rao says, take the nanotubes up in their roots, stems, and leaves, and then pass them along in their seed, afflicting the next generation. Rao is a member of a consortium operated by the National Institute of Environmental Health Sciences (NIEHS) studying the fate and health effects of nanomaterials. He collaborates with Jared Brown’s group at East Carolina University, who tests them in mice and then sends tissue samples back to Rao, whose team uses spectroscopy to detect the particles. “Mostly they seem to end up in the liver and the spleen,” Rao says, “and they cause inflammation. We’re studying how this works.”
Pollution and health risks aside, using nanotubes only as fibers to reinforce other materials—much as fiberglass and asbestos were used in the past—was a rather crude application of an elegant material. Carbon nanotubes had more on the ball than brute strength. Their extraordinary properties—electrical, optical, and thermal—hinted of a new industrial revolution, built in miniature, from the atoms up.
Into a new continent
The problem was how to take advantage of those properties. Nanotubes, and fullerenes in general, were so tiny that the usual methods and tools could seem clumsy at best. Scientists were groping their way across a new frontier, into a new continent mostly uncharted and untamed. At Clemson, Rao and his team had an advantage. They were experts in something called Raman spectroscopy, whose namesake was Sir C. V. Raman, an Indian scientist who, in 1928, first uncovered its principles by exposing organic liquid vapors to sunlight through a set of special filters. Modern Raman spectroscopy was a tool that could, in the right hands, help illuminate the realm of nano in all of its exotic detail.
But using this tool was not as simple as flipping a switch and turning a dial. There was a whole lot of science involved. The instrument scatters a powerful light, usually from a laser, that excites certain properties of molecules, shifting the energy of photons. Because the shifts reveal a material’s special modes of vibration, a scientist skilled in Raman spectroscopy can detect not only the type of material but many of its features and properties. With this powerful tool of perception, Rao’s lab began to blaze some trails across the wild frontier.
To pick up that part of the story, Rao hands me off to his right-hand man, Ramakrishna Podila, known around the lab as Rama. Rama, who earned his Ph.D. under Rao, returned to the lab as a faculty member. His projects range all over the map, but they share a common technology: Raman spectroscopy, which enabled Rama, Rao, and company to sort, select, modify, manipulate, and replicate the nanomaterials they were making or using in the lab. It also helped them reveal, to the world of science, the shape of things to come.
The long-awaited Y
In the menagerie of nanoscience, some shapes are more equal than others. One of the shapes long desired was a Y. If you had a Y, you had a junction, a potential transistor, and the makings of a circuit. It was no secret that carbon nanotubes were excellent electrical conductors and acted like rifle barrels, guiding electrons with ballistic speed. In electronics, the shorter the distance electrons must travel, the faster the device. And so, from the early days of nanoscience, people had effused about the potential for carbon to upstage silicon as a platform for supersmall microprocessors and other devices. Many a presentation to funding agencies, donors, and investors began with something like this: “Imagine a computer chip so tiny that you could park it in in a living cell…”
But none of those dreams would come true without a Y. And making a Y out of nanotubes mechanically would have been sort of like piecing them together from rolls of chicken wire: Snip off the ends of three rolls at precisely the correct angles and then weld the severed hexagons together, without wrecking the pattern, into one seamless tube with two branches. And do this on the nanoscale, working almost blind.
The Rao lab didn’t weld their Y together from pieces; they grew it, like a branching tree, using titanium as a catalyst to make branches erupt from the trunk. The results were, quite literally, electric. “Working with Prabhakar Bandaru at the University of California, San Diego, we were able to make a nanoswitch and logic gates,” Rama says. He pauses, letting that bell finish ringing. Even a tourist like me hears the implications: In electronic devices, logic gates and switches are two of those vital organs we cannot do without.
In 2005, Rao’s research group and their collaborators at UCSD published their findings in Nature Materials, announcing a dramatic breakthrough in electrical switching. Their Y transistor could serve, they wrote, as the basis for a new kind of logic device.
The capacious coil
As useful as the Y proved to be, the Rao lab did not hang a hammock on its prongs and take a rest. The team gave their nanotubes a twist. Literally. Once again, the Rao lab grew a structure it wanted, from scratch. As Rama explains it, certain metals—among them aluminum, indium, and tin—will not “wet” carbon, meaning they will not adhere. In fact, carbon has such a phobia for these metals that a nanotube will twist and turn to avoid them, growing like a vine around a tree.
That’s how the team made a coil. Some applications were obvious. Rama picks up his cell phone. “Think about the protective coating on a phone like this. The rubber coatings we have now can only take so much impact. We solve this by using nanocoils to protect devices from very high forces, by acting like coil springs in a mattress. And you don’t need a coating this thick. You only need maybe few-hundred-microns coating, about the thickness of a human hair, and it can still absorb the force.”
The Rao lab led the work on the coil, which was awarded a patent in 2010. As soon as the news of their breakthrough went public, big-time research labs came calling. Rama describes a “huge demand” from researchers at Stanford, Cal Tech, and elsewhere, all wanting samples to test in their labs. A lab at Stanford, for instance, began testing it for use in flexible electronics.
But the value of coils goes beyond the mechanical. By growing a nanotube into a coil, researchers multiplied its surface area. Think of a spiral-shaped pasta, with plenty of surface for red sauce. Nanotube coils work much the same way. They collect and retain much more charge than a simpler shape could hold.
“In a straight nanotube, you can only store energy on the surface,” Rama says. “But in a nanocoil, you can store so much more because the surface area in the coil is so much greater.
So a capacitor made of nanocoils would have a much greater storage capacity than a linear one. And the coil shape could also increase the payload of polymer coatings designed to do all kinds of jobs, from drug delivery to pathogen sensing.
The accidental antenna
Rama says the lab made the Y and the coil by design: in each case, they knew what they wanted and planned ways to grow it. But sometimes, they take advantage of a happy accident. That was the case with a nanoscale tetrapod Rama made of zinc oxide.
“I was not trying to make a tetrapod,” he says, laughing. “I was just trying to make nanowires. And it so happened that the reaction I tried gave me nanotetrapods, because of zinc oxide’s polar nature. And then we said, ‘Hey, it looks like an antenna, so why don’t we use it for sensing?’” Sensing on the nanoscale is a big idea in science, and most especially in medicine. Doctors want to see where the trouble is, in minute detail.
But many of the nanomaterials tested for imaging have had a serious limitation: They needed too much light to make them glow.
“If you increase the intensity of light, it can burn the surrounding tissues,” Rama says, “so you just want to increase the energy locally, around the area you are sensing.”
With a thin coating of gold or silver, the tetrapod’s “whiskers,” as they are called in the lab, could harbor a swarm of electrons, and thereby amplify the signal for sensing.
But a tetrapod isn’t the only shape that makes sense for sensing. The lab is also working with nanotube cantilevers, tiny diving boards anchored at one end. Each diver bounces the board a slightly different way, and Rao’s lab has found that cantilevered nanotubes resonate at various frequencies, depending on their length and diameter. The team can detect analytes—chemicals of interest in analysis—by treating the nanotube diving boards with specific chemical groups that increase sensitivity and selectivity. The sensors can detect, for example, hazardous chemicals in the tiniest amounts (parts per million).
But medicine isn’t the only endeavor in need of good sensing. The health of the environment is also at stake, and the lab pursues several technologies for detecting or cleaning up contaminants. With Tim DeVol in environmental engineering and earth sciences, the Rao lab is working on a nanoscale device for detecting radioactivity. Current technology relies on helium, which has become very scarce and expensive. The Rao lab has also created a carbon-based “bucky” sponge that separates oil from water, with a much higher capacity for soaking up an oil spill than other available products. The new sponges have a structure that resembles a parking deck, with closely knit nanotubes forming the deck and the microfibers supporting them as pillars. Bucky sponges, which have an extreme distaste for water, selectively take up oil. The sponges can be burned with no structural damage or loss of elasticity, Rao says, and can be reused over tens and hundreds of cycles.
Generation three: peeling the pencil
The lead in your pencil isn’t lead. It is most likely the mineral graphite, another form of carbon. If you had a sharpener so precise it could peel off a shaving one atom thick, you would have graphene. Graphene is a honeycomb lattice of carbon atoms with many of the same mechanical and electrical properties as the nanotube. It is just infinitesimally thin.
For Rao, graphene is the third generation, another magic carpet on which to fly a host of applications. With Rama and Frank Alexis in bioengineering (see Don’t Be Afraid), Rao’s lab has used graphene as a protective wrapper for stents, the tube-like structures inserted into arteries or other bodily passageways to repair damage or improve flow.
“The biggest problem with stents has been thrombosis, because proteins in the body interact with the stent material to create blood clots,” Rao says. “Eventually the patient has to go back to replace the stent, and the average time is about five to six years, depending on the patient. So what we have shown in the lab is that if we put one layer of graphene on this stent material we can increase the longevity of the stent and prevent the thrombosis.” The graphene, Rao says, protects the stent without adding bulk, and, like carbon nanotubes, does not break down or dissolve in the body.
Rao’s partners at East Carolina University plan to test the stent system in laboratory animals, a first step toward use in humans.
Meanwhile, the team is exploring the basic science of graphene. Last year, Rama and Rao helped resolve a scientific debate about defects in graphene, an advance that could provide insights into other questions such as: How can you fold, twist, or scroll the sheets to create and control the band gap? What happens when you fold the graphene, layer by layer? Do its electrical properties change? At what point does layered graphene begin to behave more like graphite? The answers could point to new materials and new applications.
“We have grand plans,” Rao says.
One of those plans is to take advantage of the new materials’ optical properties. Currently, the Rao lab is designing and developing thin-film optical diodes, based on graphene and buckyballs. These new optical diodes potentially could replace silicon-based technologies and result in much faster, all-optical computers.
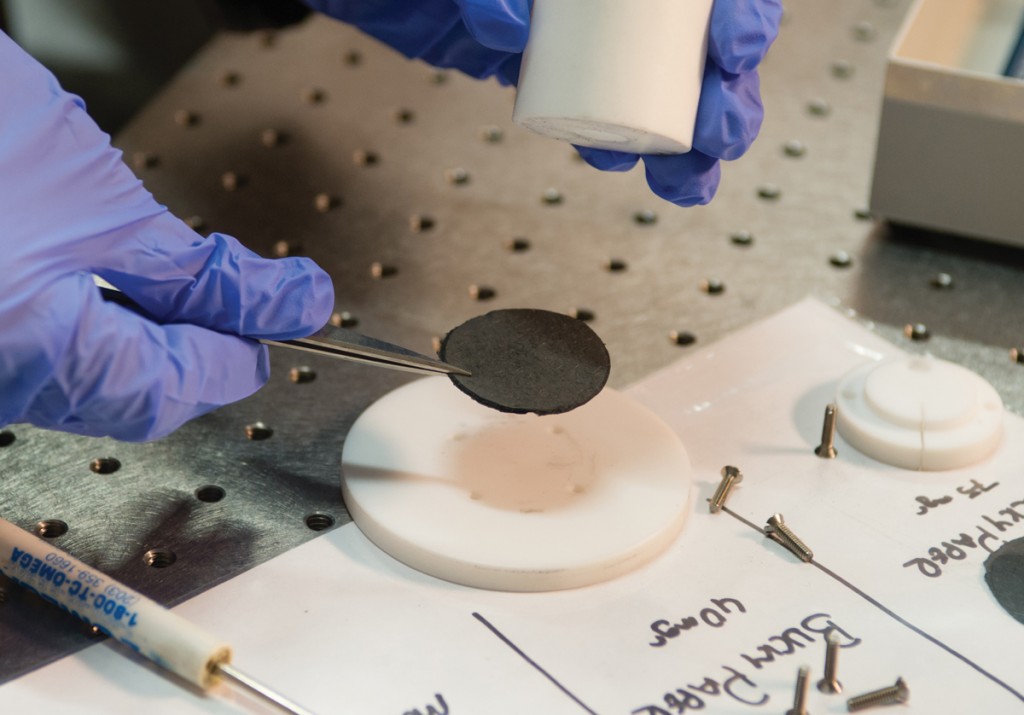
Powerful potential: An electrically conducting coiled carbon nanotube buckypaper synthesized by Mehmet Karakaya can fold, twist, and stretch without breaking. The material has potential applications in mechanical, electronic, and energy-storage technologies. Rao and Roberts are funded by the National Science Foundation to explore how engineered defects in carbon nanotube buckypaper can result in a new class of supercapacitors that have sufficient power and energy to propel automobiles. Photo by Craig Mahaffey.
The upside of being defective
With so many balls in the air, how will Rao’s team ever manage the task of building its supercapacitors and scaling them up for production? Won’t all the tantalizing tangents lead them astray?
No, Rama says. By working with multiple materials, across several scientific disciplines, the team improves the odds for creative breakthroughs, he says, because a broad frame of reference allows scientists to connect the dots and think by analogy (see Breakthrough).
“We can see those analogies because it’s the same physical laws that govern everything,” Rama says.
Just last year, a basic-science breakthrough in Rao’s lab added unexpected value to the NSF project on scalable manufacturing.
The breakthrough? A defect.
“People generally assume that defects are not good,” Rama says, “but it turns out that at the nanoscale you only have a few atoms, so if you rip one atom off in a nanotube, it makes a very big deal. We discovered that, by introducing defects, we could tune the properties. And we’re seeing that defective nanotubes can be better for capacitors and batteries.”
For one thing, a defect adds surface area, and therefore increases the capacity for electrical charge. But that’s not all. For batteries, Rama says, the goal is continuous power. But when you charge, say, a lithium-ion battery, some of the ions get clogged up inside the electrode, reducing the available energy. To solve this problem, Rama envisions something like a sprinkler head for ions. “The lithium ion should come out freely when the battery is discharging,” Rama says. “So it’s good to punch out some holes in the graphite electrode that are just about the size of lithium ions—just the right amount of holes so that the lithium ions can come out really nicely.”
Fighting carbon with carbon
At first glance, it might seem paradoxical to suppose that carbon could help decrease pollution. After all, it’s the excess of carbon, in the form of carbon dioxide, released into the atmosphere that gets most of the blame for global warming. But carbon, in the right configuration, has an almost peerless ability to conduct electricity. So, with it, we wouldn’t necessarily have to burn a carbon-based fuel such as oil, gas, or coal to put carbon to work in our energy supply. And even though the Rao lab works with a range of materials, carbon is clearly the star of the show.
“We’ve stuck with energy because we’re working with carbon, and anything related to energy you see carbon is always there,” Rao says. “Already, a lot of people are working on energy sources such as solar and wind. So we said okay, if everyone is working on how to make energy, let’s work on how to store energy. So that’s the niche we found. We want to take this to the point that, regardless of how the energy is generated, you will use this storage device.”
How is that going, so far?
“We have convinced the NSF that we have the ability to grow carbon nanotubes the way we want them, aligned in an array,” Rao says, “and that we can modify them in the right way for energy storage applications.”
Does this mean that we could use smaller batteries, extract more power from the batteries we have, and increase the use of renewable energy?
“That is the hope,” Rao says.
To get there, his team will build what they call a supercapacitor, which would store and release more energy than ordinary capacitors can handle—and last longer, too. Today’s supercapacitors typically are made with a layer of aluminum foil coated with a layer of activated carbon. But the bond between the two layers breaks down, over time.
“One of the main problems with current technology is that after the charge-discharge cycle there is delamination of the layered carbon and the aluminum,” Rao explains. “And once it is delaminated the device is useless.”
Rao and his collaborator in chemical engineering, Mark Roberts, have two plans for solving that problem. Plan A involves using aluminum foil, much like the foils in production today, but instead of applying a layer of activated carbon, the team will grow carbon nanotubes directly on the surface, essentially welding them to the foil. That could solve the delamination problem, Rao says.
But Plan B has promise, too. “Since the carbon material itself is conducting, we want to get rid of the aluminum completely and make it an all-carbon supercapacitor,” Rao says. “That way there is no need to use aluminum, and that gives more room for boosting energy storage. For Plan B, the team would buy nanotubes in bulk and form them into a paper, known as buckypaper, that would have its own electrical conductance and could also accept various polymer coatings.
Charge for cheap
For the polymers, Roberts takes the lead. “The system we’ve been using enables us to look at a variety of materials, tailored across a broad spectrum of cost versus performance,” Roberts says. “So the versatility is extremely high and rare from the standpoint of an energy system.”
The cost of storing energy, Roberts says, has been one factor limiting the widespread use of renewable energy from solar collectors or wind turbines. “Wind and solar require you to store a charge,” Roberts says. But storage is expensive. “If every renewable-energy system had to have a lithium battery pack, it would never be cost effective. So the Department of Energy is really interested in materials that can store energy for cheap.”
Roberts is testing various polymers for the supercapacitor, some of them inexpensive and easy to find.
“We’re looking at materials that have previously been dismissed as not useful for batteries because they can’t conduct electrons,” he says. “But if the carbon nanotube is providing the pathway for the electrons, then the polymer can supply the charge capacity. One of the polymers we’re testing is lignin, which is a waste product from papermaking.”
Lignin, he says, has chemical properties—redox groups—that allow it to hold a charge. When Roberts tested a coating of lignin on the layer of carbon nanotubes, the combination significantly boosted charge capacity. “So now we can have storage on the polymer and also on the interface,” he says. “We think that this lignin-carbon combination eventually could compete with lead-acid batteries. And we also have some ideas for things that could rival lithium.”
Rao says that when Roberts learned about the plan to use carbon nanotubes for a supercapacitor, Roberts quickly proposed adding a layer of polymer to increase the storage capacity.
“I knew that there would be a benefit in combining these materials,” Roberts says. “The buckypaper is an excellent conductive material, and it’s easy to process and bind things to in the lab.”
Once he began working with the combined materials, some of the test results surprised him. Roberts, who likes to push the limits, jacked up the power, exposing the polymer-coated carbon to very high voltage. “When we went to a higher voltage limit, we saw the energy storage property change,” he says. “The material was not only absorbing ions as predicted, it leveraged the presence of catalyst nanoparticles like iron that actually increased the electrical storage capacity.”
The team is building, in Clemson’s Advanced Materials Research Laboratory, what Rao calls a mini-pilot plant. The equipment feeds a spool of aluminum foil through a hot oven where the nanotubes will grow, and then into another unit for controlling defects, and then into a vat of the polymer, and then onto a take-up spool. This sort of roll-to-roll process, as Rao calls it, is common in manufacturing.
But it’s not as simple as I’m making it sound. Problems that look small in the lab can turn out to be huge on the industrial scale. The heat required to grow the nanotubes introduces various physical and chemical complications, and the mesh-like structure of the nanotube layer has to be constructed with precision— just porous enough for electrons and ions to move through it.
But Rao expects to solve these problems on schedule. “We have already made good progress,” he says.
Both ends of the market
With either Plan A or Plan B, the team expects to produce a range of energy-storage capacities, for a range of budgets and applications.
“We can satisfy both ends of the market,” Rao says. “We can make a really cheap energy-storing device or we can do a very high-end device, depending on what materials we put in there, so it allows us to cater to specific applications.”
Low-cost devices might include those in toys, health-care test kits, various disposable electronics, and low-cost batteries and capacitors. High-end applications could include electric or hybrid vehicles, power tools, and high-tech equipment of various kinds. With such a broad range of potential products and markets, the supercapacitor technology could, if it succeeded, lift whole industries on a carpet of carbon.
“We already have some industrial partners who are interested,” Rao says. KEMET Corporation, a capacitor maker based in Greenville, South Carolina, and SAI Global Technologies, Inc., a nanomaterials company based in Texas, are both working with the research team on the NSF project.
It’s this kind of possibility for real-world relevance that excites Rao most, he says. A problem solver by nature, he likes to think beyond the lab.
“Research is all fun and exciting,” he says, “but at the end of the day, it should be useful to humanity. This is the reason I get up early in the morning and come to work.”
Apparao M. Rao is the R. A. Bowen Professor of Physics in the College of Engineering and Science. He was elected to the rank of fellow by the American Physical Society (2008) and the American Academy for the Advancement of Science (2012) for his contributions to the science of carbon. Members of his laboratory include: Malcolm J. Skove, Alumni Distinguished Emeritus Professor of Physics; Ramakrishna Podila, research assistant professor; Ph.D. students Deepika Saini, Mehmet Karakaya, Jingyi Zhu, Anthony Childress, Sai Sunil Mallineni, and Chris Cortis; Herbert Behlow, a research associate; and Poonam Choudhary, an M.S. student.
Mark E. Roberts is an assistant professor of chemical and biomolecular engineering in the College of Engineering and Science. Pu-Chun Ke is an associate professor in the Department of Physics and Astronomy, College of Engineering and Science. Hong Luo is a professor in the Department of Genetics and Biochemistry, College of Agriculture, Forestry, and Life Sciences. Timothy A. DeVol is the Toshiba Professor of Nuclear Engineering in the Department of Environmental Engineering and Earth Sciences, College of Engineering and Science.
Rao, Roberts, and Podila lead Clemson’s work on the National Science Foundation (NSF) scalable manufacturing grant. They collaborate on the project with Prabhakar Bandaru, a professor of mechanical engineering at the University of California, San Diego.
Many of the projects mentioned here are patented or patent-pending technologies available for licensing and are managed by the Clemson University Research Foundation (CURF). CURF is a nonprofit corporation that facilitates the transfer of Clemson University’s intellectual property to the private sector for commercial development and societal benefit. For further information on these or other Clemson technologies, please visit www.clemson.edu/curf or email contactcurf@clemson.edu.